Glass can be made transparent and flat, or into other shapes and colours as shown in this ball from the Verrerie of Brehat in Brittany.wikipedia Glass___________________________________________________
In recent experiments, physicists have replicatedconditions of the infant universe--with startling results
By Michael Riordan and William A. Zajc
A Perfect SurpriseThe physical picture emerging from the four experiments is consistent and surprising. The quarks and gluons indeed break out of confinement and behave collectively, if only fleetingly. But this hot mélange acts like a liquid, not the ideal gas theorists had anticipated.
The energy densities achieved in head-on collisions between two gold nuclei are stupendous, about 100 times those of the nuclei themselves--largely because of relativity. As viewed from the laboratory, both nuclei are relativistically flattened into ultrathin disks of protons and neutrons just before they meet. So all their energy is crammed into a very tiny volume at the moment of impact.
Physicists estimate that the resulting energy density is at least 15 times what is needed to set the quarks and gluons free. These particles immediately begin darting in every direction, bashing into one another repeatedly and thereby reshuffling their energies into a more thermal distribution.
Evidence for the rapid formation of such a hot, dense medium comes from a phenomenon called jet quenching. When two protons collide at high energy, some of their quarks and gluons can meet nearly head-on and rebound, resulting in narrow, back-to-back sprays of hadrons (called jets) blasting out in opposite directions. But the PHENIX and STAR detectors witness only one half of such a pair in collisions between gold nuclei.
The lone jets indicate that individual quarks and gluons are indeed colliding at high energy. But where is the other jet? The rebounding quark or gluon must have plowed into the hot, dense medium just formed; its high energy would then have been dissipated by many close encounters with low-energy quarks and gluons. It is like firing a bullet into a body of water; almost all the bullet's energy is absorbed by slow-moving water molecules, and it cannot punch through to the other side.
Indications of liquidlike behavior of the quark-gluon medium came early in the RHIC experiments, in the form of a phenomenon called elliptic flow. In collisions that occur slightly off-center--which is often the case--the hadrons that emerge reach the detector in an elliptical distribution. More energetic hadrons squirt out within the plane of the interaction than at right angles to it.
The elliptical pattern indicates that substantial pressure gradients must be at work in the quark-gluon medium and that the quarks and gluons from which these hadrons formed were behaving collectively, before reverting back into hadrons. They were acting like a liquid--that is, not a gas. From a gas, the hadrons would emerge uniformly in all directions.
This liquid behaviour of the quark-gluon medium must mean that these particles interact with one another rather strongly during their heady moments of liberation right after formation. The decrease in the strength of their interactions (caused by the asymptotic freedom of QCD) is apparently overwhelmed by a dramatic increase in the number of newly liberated particles.
It is as though our poor prisoners have broken out of their cells, only to find themselves haplessly caught up in a jail-yard crush, jostling with all the other escapees. The resulting tightly coupled dance is exactly what happens in a liquid. This situation conflicts with the naive theoretical picture originally painted of this medium as an almost ideal, weakly interacting gas. And the detailed features of the elliptical asymmetry suggest that this surprising liquid flows with almost no viscosity. It is probably the most perfect liquid ever observed.
The Emerging Theoretical PictureCalculating the strong interactions occurring in a liquid of quarks and gluons that are squeezed to almost unimaginable densities and exploding outward at nearly the speed of light is an immense challenge.
One approach is to perform brute-force solutions of QCD using huge arrays of microprocessors specially designed for this problem. In this so-called lattice-QCD approach, space is approximated by a discrete lattice of points (imagine a Tinkertoy structure). The QCD equations are solved by successive approximations on the lattice.
Using this technique, theorists have calculated such properties as pressure and energy density as a function of temperature; each of these dramatically increases when hadrons are transformed into a quark-gluon medium. But this method is best suited for static problems in which the medium is in thermodynamic equilibrium, unlike the rapidly changing conditions in RHIC's mini bangs. Even the most sophisticated lattice-QCD calculations have been unable to determine such dynamic features as jet quenching and viscosity. A
lthough the viscosity of a system of strongly interacting particles is expected to be small, it cannot be exactly zero because of quantum mechanics. But answering the question "How low can it go?" has proved notoriously difficult.
Remarkably, help has arrived from an unexpected quarter: string theories of quantum gravity. An extraordinary conjecture by theorist Juan Maldacena of the Institute for Advanced Study in Princeton, N.J., has forged a surprising connection between a theory of strings in a warped five-dimensional space and a QCD-like theory of particles that exist on the four-dimensional boundary of that space [see "The Illusion of Gravity," by Juan Maldacena; Scientific American, November 2005].
The two theories are mathematically equivalent even though they appear to describe radically different realms of physics. When the QCD-like forces get strong, the corresponding string theory becomes weak and hence easier to evaluate. Quantities such as viscosity that are hard to calculate in QCD have counterparts in string theory (in this case, the absorption of gravity waves by a black hole) that are much more tractable.
A very small but nonzero lower limit on what is called the specific viscosity emerges from this approach--only about a tenth of that of superfluid helium. Quite possibly, string theory may help us understand how quarks and gluons behaved during the earliest microseconds of the big bang.
Future ChallengesAstonishingly, the hottest, densest matter ever encountered far exceeds all other known fluids in its approach to perfection. How and why this happens is the great experimental challenge now facing physicists at RHIC. The wealth of data from these experiments is already forcing theorists to reconsider some cherished ideas about matter in the early universe.
In the past, most calculations treated the freed quarks and gluons as an ideal gas instead of a liquid. The theory of QCD and asymptotic freedom are not in any danger--no evidence exists to dispute the fundamental equations. What is up for debate are the techniques and simplifying assumptions used by theorists to draw conclusions from the equations.
To address these questions, experimenters are studying the different kinds of quarks emerging from the mini bangs, especially the heavier varieties. When quarks were originally predicted in 1964, they were thought to occur in three versions: up, down and strange.
With masses below 0.15 GeV, these three species of quarks and their antiquarks are created copiously and in roughly equal numbers in RHIC collisions. Two additional quarks, dubbed charm and bottom, turned up in the 1970s, sporting much greater masses of about 1.6 and 5 GeV, respectively. Because much more energy is required to create these heavy quarks (according to E = mc2), they appear earlier in the mini bangs (when energy densities are higher) and much less often.
This rarity makes them valuable tracers of the flow patterns and other properties that develop early in the evolution of a mini bang.
The PHENIX and STAR experiments are well suited for such detailed studies because they can detect high-energy electrons and other particles called muons that often emerge from decays of these heavy quarks. Physicists then trace these and other decay particles back to their points of origin, providing crucial information about the heavy quarks that spawned them. With their greater masses, heavy quarks can have different flow patterns and behavior than their far more abundant cousins. Measuring these differences should help tease out precise values for the tiny residual viscosity anticipated.
Charm quarks have another characteristic useful for probing the quark-gluon medium. Usually about 1 percent of them are produced in a tight embrace with a charm antiquark, forming a neutral particle called the J/psi. The separation between the two partners is only about a third the radius of a proton, so the rate of J/psi production should be sensitive to the force between quarks at short distances.
Theorists expect this force to fall off because the surrounding swarm of light quarks and gluons will tend to screen the charm quark and antiquark from each other, leading to less J/psi production. Recent PHENIX results indicate that J/psi particles do indeed dissolve in the fluid, similar to what was observed earlier at CERN, the European laboratory for particle physics near Geneva [see "Fireballs of Free Quarks," by Graham P. Collins, News and Analysis; Scientific American, April 2000]. Even greater J/psi suppression was expected to occur at RHIC because of the higher densities involved, but early results suggest some competing mechanism, such as reformation of J/psi particles, may occur at these densities. Further measurements will focus on this mystery by searching for other pairs of heavy quarks and observing whether and how their production is suppressed.
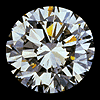
Another approach being pursued is to try to view the quark-gluon fluid by its own light. A hot broth of these particles should shine briefly, like the flash of a lightning bolt, because it emits high-energy photons that escape the medium unscathed. Just as astronomers measure the temperature of a distant star from its spectrum of light emission, physicists are trying to employ these energetic photons to determine the temperature of the quark-gluon fluid. But measuring this spectrum has thus far proved enormously challenging because many other photons are generated by the decay of hadrons called neutral pions. Although those photons are produced long after the quark-gluon fluid has reverted to hadrons, they all look the same when they arrive at the detectors.
Many physicists are now preparing for the next energy frontier at the Large Hadron Collider (LHC) at CERN. Starting in 2008, experiments there will observe collisions of lead nuclei at combined energies exceeding one million GeV. An international team of more than 1,000 physicists is building the mammoth ALICE detector, which will combine the capabilities of the PHENIX and STAR detectors in a single experiment. The mini bangs produced by the LHC will briefly reach several times the energy density that occurs in RHIC collisions, and the temperatures reached therein should easily surpass 10 trillion degrees. Physicists will then be able to simulate and study conditions that occurred during the very first microsecond of the big bang.
The overriding question is whether the liquidlike behavior witnessed at RHIC will persist at the higher temperatures and densities encountered at the LHC. Some theorists project that the force between quarks will become weak once their average energy exceeds 1 GeV, which will occur at the LHC, and that the quark-gluon plasma will finally start behaving properly--like a gas, as originally expected. Others are less sanguine. They maintain that the QCD force cannot fall off fast enough at these higher energies, so the quarks and gluons should remain tightly coupled in their liquid embrace. On this issue, we must await the verdict of experiment, which may well bring other surprises.
___________________________________________________
Further sources & reading:
Color Glass Condensate by Plato
Attributes of Superfluids by Plato
The Right Spin for Neutrino Superfluid by Plato
Supersolid, Quantum Crystal, A Bose-Einstein Condensate in Solid AIP
The right spin for a neutrino superfluid CERN Courier
_____________________________________________________________________
_____________________________________________________________________
Labels: Particle Physics, QGP